
ð
WORLD’s
FIRST and
ONLY ï
One of Most Significant Inventions in a Century
!
FluAWAYTM
Anti-Flu Herbal Remedy
Possible Mechanism of Action
Review of Pathogens Detected in Patient with Pneumonia Symptoms
Manifestation
Pneumonia is
a leading cause of hospitalization worldwide and carries significant
morbidity and mortality that differ according to the underlying etiology.[8]
In general,
viral pneumonia is considered less severe compared to bacterial pneumonia.
[11]
·
Bacterial
pneumonia
is a common contributor to severe outcomes of influenza. Epidemiological
data suggest that the incidence, severity and associated bacterial pathogens
differ between epidemics and by geographical location within epidemics. Data
from animal models demonstrate precisely these differences in both viral and bacterial
strains alter the incidence and outcomes of pneumonia. For influenza
viruses, evolutionary changes (as specific virulence factors) appear to alter
the ability of viruses within particular lineages to prime the host for
secondary bacterial infection. Although bacterial strains differ
considerably in their disease potential for pre-dispositioning of viral co-infection, the
bacterial virulence factors underlying this finding are currently unknown.
The hypothesis, that geographical variation do exist in the prevalence of
bacterial strains expressing factors that
enable efficient disease-causing potentiation during viral epidemics, should be
considered as one explanation for the regional differences in disease severity.
This would have further implications for surveillance, vaccine development, and the
conduct of clinical trials for the prevention or treatment of pneumonia.[36]
The
significant role of bacterial co-infection in past pandemics, and in
seasonal influenza, has been documented. [11,21.38,40,44]
During the 1918–1919 influenza pandemic, the microorganisms most frequently
recovered from the sputum, lung and blood of infected patients were Haemophilus
influenzae, Streptococcus
pneumoniae,
Streptococcus pyogenes and Staphylococcus
aureus.[11,38] In most cases, lung samples
taken from patients dying during the 1918–1919 pandemic demonstrate
bacteriologic and histopathologic evidence of severe acute bacterial
pneumonia.[38] During
the Asian and Hong Kong influenza pandemics of 1957 and 1968, S. pneumoniae,
H. influenza
and S. aureus were
most frequently isolated from patients with bacterial pneumonia.
[3,34,40,44] In 1957, S. aureus and
S. pneumoniae were
isolated from 59% and 15% of lung cultures, respectively.[21]
Most deaths from S. aureus were
observed in adolescents and young adults.
During the
2009 influenza pandemic, initial clinical descriptions reported a severe
respiratory illness with rapid progression to acute respiratory distress
syndrome (ARDS).[23] Co-detection
of clinically relevant bacteria with influenza A/H1N109 was infrequent, yet
31% of patients admitted to ICU had a clinical diagnosis of sepsis and 95%
received antibiotics.[25] Bacterial
co-infection was documented using histopathological, immunohistochemical and
molecular evidence in 22 of 77 (28·6%) subjects with fatal pandemic
influenza A/H1N1 2009 infection. Streptococcus
pneumoniae, S. pyogenes
and S. aureus
were the predominant
bacterial pathogens detected.
Bacterial or
viral co-infection has complicated one in four patients admitted to ICU with
severe influenza A infection. Co-infection
was identified in 23·3–26·9% of patients with severe influenza A infection. Staphylococcus
aureus infection was the most
frequent bacterial co-infection followed by
Streptococcus
pneumoniae and Haemophilus
influenzae. Patients with co-infection were younger [mean
difference in age = 8·46 years (95% CI: 0·18–16·74 years)], less likely to
have significant co-morbidities (32·0% versus 66·2%, P = 0·004)
and less-frequently obese [mean difference in body mass index = 6·86
(95% CI: 1·77–11·96)] compared to those without co-infection –
suggesting at increased personal mobility as a possible contributing factor
for microbial co-infection; which seems somethat logical consequience. Empiric
antibiotics with staphylococcal activity should be strongly considered in
all patients with severe influenza A infection.
Overall, twenty-seven studies including 3215 participants met all inclusion criteria
taken from publication data.
Common etiologies were defined from a subset of eight articles. There was
high heterogeneity in the results (I2
=
95%), with reported coinfection rates ranging from 2% to 65%.
The most common coinfecting species were
Streptococcus pneumoniae
and
Staphylococcus aureus,
which accounted for 35% (95% CI, 14%–56%)
and 28% (95% CI, 16%–
40%) of infections, respectively; a
wide range of other pathogens caused the
remaining infections.
The frequency of co-infection in the published studies, included in this
review, suggests that, although providers should consider possible bacterial
coinfection in all patients hospitalized with influenza, they should not
assume that all patients are coinfected and ensure to properly treat underlying
viral processes. Further, high
heterogeneity suggests that additional large-scale studies are needed to
better understand the etiology of influenza co-infection with bacteria.
[29]
Researchers from San Diego confirmed that
infections with flu virus and with Haemophilus influenzae can be
lethal when the flu infection precedes the bacterial one. That
was true even for infections that, if experienced separately, would not have
been lethal; it was the synergy of the two
infections, flu first followed by the bacterial infection, that caused the
high mortality rate. In
1968, much of the excess mortality was attributed to the increased incidence
of bacterial pneumonia: a three-fold increase in the incidence of
staphylococcal pneumonias and strong correlation between staphylococcal
pneumonia and prior influenza infection was observed.[44] The
results may not be directly applicable to human medicine (Do you all know
the old flu-research saying, “Mice lie and ferrets mislead”?), but they are
an important indicator of both the seriousness of bacterial infection after
flu, and also of the potential vulnerability of even healthy beings to that
double-punch[33].
·
Viral
pneumonia. In the past decade the mortality related to Viral pneumonia
has substantially increased because of the emergence of the new
respiratory viruses such as influenza-A H1N1, the Middle East respiratory
syndrome-coronavirus (MERS-CoV) and the Coronavirus Disease of 2019
(COVID-19).[9]
Moreover,
high mortality was reported in previously healthy middle-aged patients
during influenza-A H1N1 pandemic infection in 2009.[35] Reports
from the Southern-hemisphere countries suggested that the hospitalization
rates secondary to influenza-A H1N1 ranged from 23.6% to 30.6%; among them,
11.7% to 18.5% were admitted to the Intensive Care Units (ICUs) with
mortality rate ranged from 16% to 41%.17 Noteworthy, according to
the last update from the center for diseases control (CDC) on February 2014,
pneumonia and influenza were the cause of death in 8.4% of all deaths in the
United States, of which 34% occurred in persons aged ≥65 years, 62% in
persons aged 25–64 years, and 4% in persons younger than 24 years old.
[13]
As by July
2017, the World Health Organization (WHO) had received reports from 27
countries of 2040 cases of laboratory-confirmed MERS and at least 677
related deaths representing case fatality of 35%.[52]
The course of the disease
has been described to vary from asymptomatic viral illness to dramatically
fatal respiratory failure and ARDS. Gastrointestinal and renal involvement
have also been reported in one-third and one-half of the cases, respectively[2]
(Note the high similarity to the
COVID-19 infection observations outlined further below). In Saudi Arabia, MERS-CoV virus was first
reported in 2012, and between September 1, 2012, and June 15, 2013, there
have been 47 laboratory-confirmed cases (46 adults and one child) with a
fatality rate of 60%[5]Assiri et
al., had reported the epidemiological characteristics of the
MERS-CoV in Saudi Arabia either as a community cases running in clusters
within the families or among healthcare workers with or without direct camel
contacts.[5] During June–August, 2015, a second MERS-CoV outbreak
occurred with a total of 130 MERS-CoV cases detected. Overall, 96 patients
(74%) required hospitalization, of them 63 (66%) required intensive care
management, and the fatality rate was 53%.[7]
Viral
pneumonia remains a major health problem, particularly after emergence of
new respiratory viruses, including influenza, and have received more
attention compared to other causes of pneumonia after the emergence of the
new H1N1 and MERS-CoV.
The fatality
rate was high particularly among cases associated with MERS-COV
infection. The most important predictors of death among these patients were
old age, male sex, and associated comorbidities.
A similar finding was also reported in studies from other Middle-East
countries including UAE, Qatar, Oman, Jordan, Kuwait, and Yemen.[10]
On the contrary, H1N1 influenza pneumonia affects patients at
a slightly younger age group.[4,16,15,31]
It was noted
during most pandemics that the age distribution of severe influenza-related
pneumonia exhibits a U-shaped pattern, with young and the elderly patients
were most frequently affected.[3,45] In this cohort, males were
more likely to be infected. This is consistent with a similar finding
reported by others[4,10,15,16,30], although a recent report
showed more MERS-CoV infection among female healthcare workers.[3]
The most
common radiological finding in this study was bilateral lung infiltrate and
that was mostly observed among MERS-CoV (94.1%) and H1N1 (86.7%) associated
infections.
The
pattern of interstitial infiltrates with patchy distribution on chest
radiograph was sometimes useful in differentiating viral from bacterial
community-acquired pneumonia.[48] These specifics, however,
were not universal. Similarly, the presence of pleural effusion predicts
bacterial infection,[22] and that has not been reported in any of the
studied cases.
·
Coronavirus Disease 2019
(COVID-19) infection, caused
by severe acute respiratory syndrome coronavirus-2 (SARS-CoV-2), is
spreading globally and poses a major public health threat.[56]
No effective treatment has yet been established and severe morbidity and
mortality have been reported to be higher in the elderly and patients with
underlying diseases.[32]
COVID-19 often starts with non-specific
upper respiratory tract symptoms, making it difficult
to distinguish from those of other diseases.[9]
In particular, influenza
virus infections may present similar symptoms as those of COVID-19. The
current lack of clinical knowledge about COVID-19 might, therefore, lead to
bias and missed diagnoses in cases of co-existing infections. In one
hospital, was encountered a case of influenza
A virus and SARS-CoV-2 co-infection, which allowed to analyze the
overlapping clinical course of these two viral infections.[47]
COVID-19
and
Influenza co-infection can occur in patients and can present with similar
symptoms.[6,28,30,41,49,53,54]
It
is essential to recognize the co-infections as some can be treated with
antibiotics and antivirals. We already have treatments for influenza, but
while multiple drugs are
being investigated for COVID-19, none have been approved for treatment so
far.
COVID-19 can
initially present with minor symptoms such as fever with or without chills,
dry cough, shortness of breath, fatigue, muscle aches, sore throat,
confusion, headache and rhinorrhea. The lung is the main organ affected,
which can result in respiratory failure. The disease can also present with
atypical symptoms such as nausea, vomiting and diarrhea.[8]
Influenza in
the USA occurs mainly during winter, and the burden of disease is determined
by several factors, including the effectiveness of
the vaccine that season, the characteristics of the circulating viruses, and
how long the season lasts. According to CDC estimates, during
the 2018–2019 season symptomatic influenza occurred in approximately 35
million patients[26],
which resulted in approximately 16 million hospital visits and approximately
500,000 hospitalizations with 34,000 deaths[14].
The most common
symptoms of influenza are fever, cough,
shortness of breath, fatigue, headache, myalgia and
arthralgia, similar to those of COVID-19.
COVID-19 can
simultaneously present with other infections such as influenza, and it can
be hard to distinguish the symptoms of the two conditions from each other.
However, there are differences and these are summarized in
Table 3.[8]
A study by Xing et al. analyzed common
respiratory pathogens presenting as co-infections with COVID-19 from
Quingdao and Wuhan. This report identified IgM antibodies to at least one
respiratory pathogen in 80% and 2.6% of the patients from Quingdao and
Wuhan, respectively.
Influenza A,
influenza B, followed
by
Mycoplasma
and
Legionella,
were the most common respiratory pathogens detected.[55]
In a study
from Wuhan, five of 115 patients were co-infected with COVID-19 and
influenza.
Most of these patients presented with fever, cough and shortness of breath.
All of the co-infected patients presented with pharyngeal pain.
Only one of the co-infected patients developed acute respiratory distress
syndrome and required non-invasive ventilation.
Finally,
routine testing for newly emergent viruses may be warranted for adults who
have been hospitalized with pneumonia.
Table 1.
Differences between influenza and COVID-19 diseases.
|
Influenza
|
COVID-19
|
Asymptomatic or symptomatic
|
Patients can be asymptomatic due to herd immunity
|
Most patients develop symptoms within 2 days of infection
|
Viral shedding
|
5–10 days
|
Up to 14 days or even longer
|
Severity of illness
|
Majority of infections are mild to moderate
|
Severe illness can occur
|
Mortality
|
Less than 1%
|
3–4%
|
Vaccines
|
Vaccines available; efficacy varies from season to season
|
No vaccine available, clinical trials in progress
|
Treatment
|
Oseltamivir Zanamivir Peramivir Baloxavir
|
No treatment available, clinical trials in progress
|
Acute respiratory distress syndrome
|
Less common
|
More common
|
FluAWAYTM
Possible Mechanism of Action
The mechanism
by which FluAWAYTM eliminates the cold/pheumonia symptoms is still unknown. Since
lipid-based communication and trans-membrane signaling is the first
evolutionary mean of communication among species, we speculate that FluAWAYTM
component(s) affects (reduce or modify) the cell receptors to which the
cold- and pneumonia-causing viruses and/or microbes attaches to invade the
body; or somehow, in addition, it assists the immune system in eliminating the infection –
since the cold/pneumonia disease is generally caused by simultaneous
multi-microbial and multi-viral co-infection. We favor the first hypothesis
due to the instant effect observed after FluAWAYTM
application
– while assisting the immune system may play role at the later
stages of disease elimination.
It
is well-known that the cell membrane provides a reservoir from which
biologically active signaling lipids, or their precursors, are released by a
variety of hydrolytic enzymes. Research has shown, that lipid‐transfer
proteins are capable of influencing the outcome of host–pathogen
interactions. Lipid modifications target signaling proteins and
microbial elicitors to cell membranes where defense signaling is initiated.[27]
Another class of biologically active compounds,
flavonoids
are metabolites synthesized mainly by plants. They can act as chemical
messengers, physiological regulators, and cell cycle inhibitors.
Laboratory studies indicate that
flavonoids have effects on isolated cells or cell cultures in vitro
and have been shown in preliminary research to have anti-inflammatory
mechanisms including
inhibition of gene
transcription, expression and activity of inflammatory enzymes, as well as
secretion of anti-inflammatory mediators.[61]
The plants with flavonoids as their major constituents, for example, can
inhibit Helicobacter pylori infection and are used as anti-peptic
ulcer disease.[62]
Flavonoids have also been implicated to modify intracellular
signaling pathways in immune cells,[63]
or in brain cells after a
stroke.[64]
Another
reasonable hypothesis speculates, that, because the nose is the normal
ecological location where both non-pathogenic and pathogenic strains of
microbes like Streptococcus reside normally[37], the
application of FluAWAYTM inside the nose bringing
plant-derived ani-microbial substances serves as a straight-to-the-source cut-off
factor preventing the spread of the human pathogens wide inside the body. The
immediate relief action resulting from the application of FluAWAYTM
inside the nose supports this hypothesis.[60]
Our
tests over a 10-year period show that FluAWAYTM acts in a
different way than the antibiotics. For example: The flu invasion usually
manifests its symptoms starting from the nose, then moving to the throat,
and then – the lungs and the entire body. The antibiotic-mediated
relieve-symptoms manifest the same way: relieving first the nose, then the
throat and the entire body at last. The FluAWAYTM,
however, relieves the lungs first (while initially easing the nose-affecting
symptoms), then the throat and at last completely removing the nose
irritation.
How to apply FluAWAYTM creme
Place FluAWAYTM
creme amount equal to the size of pea-bean on the top of a cotton-swab stick
or the back of a finger nail. Introduce it high-up into each nozzle cavity and apply it
evenly up there. Breed-in sharply by closing the opposite nozzle to depose
the creme high into the sinuses.
Incidental swallowing it is
of no concern – in fact the
remedy (prepared as a solution) can also be used to calm stomach infections
and cases of diarrhea.
At
best, we recommend to use FluAWAYTM immediately after the
occurrence of the first cold/pneumonia symptoms. This provides for fastest
prevention of the infection with introducing tiniest amounts of the crème;
if so – no flu infection will further develop in person’s body. Depending on
the stage of infection and the personal specifics, the flu-symptoms
elimination may be as fast as 1-2 applications and within few hours only
(overnight application is highly recommended),
or to take up to 3 days.
Although FluAWAYTM may be used for up to 5 days or more, if
desired, usually 3 days with 3-5 daily applications are enough for most
people to eliminate the pneumonia symptoms. In severe cases, FluAWAYTM
crème can be applied every hour during the first day, but usually no such a
frequent application is needed after 24 hours of use. However,
in cases of Covid-19
or similar coronavirus infection treatment, we strongly recommend hourly
nasal applications until diminished symptomatic is achieved completely.
When
FluAWAYTM is applied before meetings in a closed space
with peoples (possible) already having a flu – no single case of catching a
flu out of hundreds of occasions has been observed previously.
NOTE,
that we have no data on how FluAWAYTM usage may affect
pregnancy or infants. Although side-effects were never detected and are not
expected due to the negligible
herbal amounts deposited, allergy effects might be possible. Therefore, as a precaution, we
recommend allergy skin test to be performed for 24 hours before using FluAWAYTM
for a first time.
Review of Bio-Communication Pathways in Human and animals
Lipids
are not just used as a passive component of membranes, or as a source of
stored energy. They are involved in the process of signal transduction at
the cell membrane, a process by which the interior components of the cell
respond to a signal external to the cell, allowing the cell to respond to
their local environment. Usually a chemical signal on the outside of the
cell is the "primary messenger" that causes the cell to respond. Normally the
chemical transmitter of information does not get into the cell. Rather it
binds to surface receptors on the cell membrane surface. Somehow, the cells
senses that a ligand is bound to the outside. Enzymes within the
membrane or at the intracellular surface of the membrane lipid bilayer are activated.
Many of these enzymes cleave lipids located in the membrane. The cleaved fragments
of the lipid molecules then serve as intracellular signals or "secondary
messengers", which can bind to intracellular enzymes to activate
intracellular processes. The following diagram (Fig. 1) shows some of the lipid
mediators which are generated by the process and signal the cell to respond.
These are the bio-informational pathways served by lipid-associated
functions:
·
Lipids act as extracellular and intracellular messengers to control cell
fate in normal physiology and disease. When deregulated, lipid signaling
contributes to inflammation and cancer, metabolic, cardiovascular (blood
pressure, etc.) and degenerative disease.
·
Signals such as growth factors, cytokines and chemokines, but also
constituents of nutrients, modulate the activity of lipid-modifying enzymes:
phosphoinositide 3-kinase (PI3K), sphingosine kinase (SphK), phospholipase C
(PLC) and PLD function upstream of the activation of phospholipase A2
(PLA2), prostaglandin H2 synthase (PGH2S) and 5-lipoxygenase (5-LO), which
are required for eicosanoid release.
·
Eicosanoids such as prostaglandins and leukotrienes have been known to have
a role in inflammation. Recently, their extracellular action on G
protein-coupled receptors (GPCRs) has been deciphered, and intracellular
binding partners of eicosanoids have also been identified, which opens new
possibilities for more selective drug-targeting strategies in inflammation
and cancer.
·
Inflammation and cancer are both characterized by excess activation of PI3K
and SphK pathways, which enforces growth-factor-receptor signaling, cell
growth and survival, cell motility and degranulation. This overrides the
pro-apoptotic actions of ceramide and sphingosine.
·
Although the nutritional energy supply normally increases insulin and lipid
signaling through PI3K, excess circulating fatty acids induce long-term
insulin resistance and function as pro-inflammatory agents. This occurs
through signaling via the Toll-like receptor TLR4 and through endoplasmic
reticulum stress promoted by intracellular lipid accumulation. It results in
the production of reactive oxygen species and the activation of inflammatory
kinase pathways (PKC, IKKβ), terminating the relay of signals through
insulin receptor substrate (IRS).
·
Lipid-modifying enzymes, pathways and their downstream targets, including
nuclear receptors and lipid-binding proteins, form a complex signaling
network. Although many of these lipid mediators emerge from the same
membranes, they are usually studied in isolation. Here, we present an
integrated overview of lipid signaling in disease and highlight nodes of
lipid signaling pathway interaction, and discuss emerging strategies for
therapeutic interventions.
·
Steroids are
another class of lipid molecules,
identifiable by their structure of four fused rings. Although they do not
resemble the other lipids
structurally,
steroids are
included in lipid category
because they are also hydrophobic and insoluble in water.
Recently, steroids from starfish (similar to bile acids in the human stomach)
tested against normal mouse epidermal, human breast cancer and colorectal
carcinoma cells, the steroids were demonstrated to have a pronounced
anticancer effect.[59]
Another
recent report evidences that
glucocorticoids may modulate inflammation-mediated lung injury and thereby
reduce progression to respiratory failure and death.[60]
A pheromone is
a secreted or excreted chemical factor
that triggers a social response in members of the same species
and/or across species.
Pheromones are chemicals capable of acting like hormones outside the body of
the secreting individual, to impact the behavior of the receiving
individuals. There are alarm pheromones, food trail pheromones, sex
pheromones, and many others that affect behavior or physiology.
Pheromones are used from basic
unicellular prokaryotes to
complex multicellular
eukaryotes. Their
use among insects has
been particularly well documented. In addition, some
vertebrates,
plants and ciliates communicate
by using pheromones. Pheromones are also sometimes classified as
ecto-hormones. These chemical messengers are transported outside of the body
and affect
neurocircuits,
including the autonomous
nervous system with hormone or cytokine-mediated
physiological
changes,
inflammatory
signaling,
immune system changes
and/or behavioral change in the recipient.
In reptiles, amphibia and
non-primate mammals pheromones are detected by regular
olfactory membranes,
and also by the vomeronasal
organ (VNO),
or Jacobson's organ, which lies at the base of the nasal
septum between
the nose and mouth and is the first stage of the accessory
olfactory system.
While the VNO is present in most amphibia, reptiles, and non-primate
mammals, it
is absent in birds,
adult catarrhine monkeys
(downward facing nostrils, as opposed to sideways), and apes. An
active role for the human VNO in the detection of pheromones is disputed;
while it is clearly present in the fetus it
appears to be atrophied,
shrunk or completely absent in adults. Nevertheless, the VNO is only one way
of detecting intra-species signaling – others are the direct delivery into
the body via skin- and nasal pathways wherefrom they can enter the internal
body communication transport network being deposited into the cerebral
liquid, blood and lymph and be delivered to their cellular target where they
exercise their molecular communication purpose.
Practically in all species, the pheromones are associated with a
lipid molecular content in order to become a biologically active substance –
steroids, aliphatic acids, lipidic function-stimulators and others. Thereby
all inter-cellular and inter-species signaling and communications are based
upon lipid (i.e. fatty-acids) molecular association and assistance.
Recently, fatty acid amides have been shown to be potent
mediators of neurological processes.[57] In one interesting
experiment, sheep were sleep deprived. Reasoning that the brain might
release a biochemical signal into cerebrospinal fluid to induce sleep,
scientists at Scripps removed some of this fluid and isolated a substance
that was not found in rested sheep. On analysis, the structure was shown to
be an amide of oleic acid. Oleylethanolamide has been shown to bind to the
peroxisome-proliferator-activated receptor-a (PPAR-a) which resides in the
nucleus. This ligand, by affecting gene transcription, appears to regulate
body weight and the feeling of fullness after eating (satiety) as it leads
to reduced eating.
In an analogous fashion, people have sought the natural
neurotransmitter, which binds to the same receptor in the brain as THC, the
active ingredient of marijuana. This was found several years ago and was
shown to be the amide of arachidonic acid, called anandamide.
Figure 1: Lipid Signaling in disease
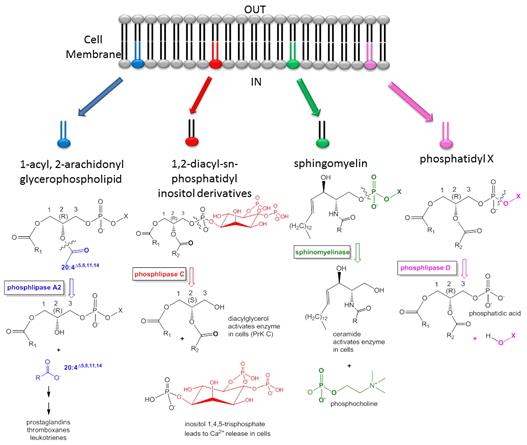
Figure
2: Lipids - Mediators in Signal Transduction
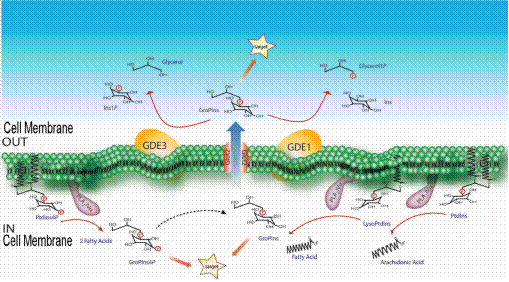
Figure
3: Schematic representation of the
Glyce-rophosphoinositols
metabolism (from:
Patrussi, L., Mariggiò, S., Corda, D and Baldari, C.T. The glycerol-
phosphoinositols: from lipid metabolites to modulators of T-cell signaling.
Front. Immunol., 29 July 2013| (https://doi.org/10.3389/fimmu.2013.00213)
·
Another class of biologically active compounds,
flavonoids
are metabolites synthesized mainly by plants. The general structure of
flavonoids is a 15-carbon skeleton, containing 2 benzene rings connected by
a 3-carbon linking chain. They
can act as chemical messengers, physiological regulators, and cell cycle
inhibitors.
Laboratory studies indicate that flavonoids have effects on isolated cells
or cell cultures in vitro.
Inflammation has been implicated as a possible origin of numerous local and
systemic diseases, and flavonoids have been shown in preliminary research
to have anti-inflammatory mechanisms including
inhibition of gene transcription, expression and activity of inflammatory
enzymes, as well as secretion of anti-inflammatory mediators.[61]
The plants with flavonoids as their major constituents, for example, can
inhibit Helicobacter pylori infection and are used as anti-peptic
ulcer disease.
[62]
Flavonoids have also been implicated to
modify intracellular signaling pathways in immune cells,[63]
or in brain cells after a stroke.[64]
Review
of Microbial and Plant Communication Pathways
The yeast Saccharomyces
cerevisiae produces pheromones (that
have strong similarities to peptide hormones in mammals) which seem to
participate in the mating process by binding to cell receptors
But
in the early 1980s, the University of Washington zoologist David Rhoades was
finding evidence that plants actively defend themselves against insects.
Masters of synthetic biochemistry, they manufacture and deploy chemical and
other weapons that make their foliage less palatable or nutritious, so that
hungry bugs go elsewhere.
Plant
Pheromones are chemicals released by an organism into its environment
enabling it to communicate with other members of its own species. There are
alarm pheromones, food trail pheromones, sex pheromones, and many others
that affect behavior or physiology.
Plant
semiochemicals are known to produce a wide range of behavioral responses in
insects. Some insects sequester or acquire host plant compounds and use them
as sex pheromones or sex pheromone precursors. Other insects produce or
release sex pheromones in response to specific host plant cues, and
chemicals from host plants often synergistically enhance the response of an
insect to sex pheromones. Plant volatiles can also have inhibitory or
repellent effects that interrupt insect responses to pheromones and attract
predators and parasitoids to the attacking species after herbivory injury.
Green
leaf volatiles interrupt aggregation pheromone response in bark infesting
pines.
It’s
now well established that when bugs chew leaves, plants respond by releasing
volatile organic compounds into the air.
Accoding
to Richard Karban, an ecologist at the University of California, Davis,
40
out of 48 studies of plant communication confirm that other plants detect
these airborne signals and ramp up their production of chemical weapons or
other defense mechanisms in response. “The evidence that plants release
volatiles when damaged by herbivores is as sure as something in science can
be,” said Martin
Heil,
an ecologist at the Mexican research institute Cinvestav Irapuato. “The
evidence that plants can somehow perceive these volatiles and respond with a
defense response is also very good.”
More broadly, the possibility that plants share information raises
intriguing questions about what counts as behavior and communication — and
why organisms that compete with one another might also see fit to network
their knowledge.
Scientists are also exploring how the messages from these signals
might spread. Just a few months ago, the plant signaling pioneer Ted Farmer of the University of Lausanne discovered
an almost entirely unrecognized way that plants transmit information — with
electrical pulses and a system of voltage-based signaling that is eerily
reminiscent of the animal nervous system. “It’s pretty spectacular what
plants do,” said Farmer. “The more I work on them, the more I’m amazed.”
Ted
Farmer’s team placed
microelectrodes on the leaves and leaf stalks of Arabidopsis thaliana (a model organism, the plant
physiologist’s equivalent of a lab rat) and allowed Egyptian cotton
leafworms to feast away. Within seconds, voltage changes in the tissue
radiated out from the site of damage toward the stem and beyond. As the
waves surged outward, the defensive compound jasmonic acid accumulated, even
far from the site of damage. The genes involved in transmitting the
electrical signal produce channels in a membrane just inside the plant’s
cell walls; the channels maintain electrical potential by regulating the
passage of charged ions. These genes are evolutionary analogues to the
ion-regulating receptors that animals use to relay sensory signals through
the body. “They obviously come from a common ancestor, and are deeply
rooted,” Farmer said. “There are lots of interesting parallels. There are
far more parallels than differences.”
Ted
Farmer, then a postdoc in the Washington State University lab of renowned
plant hormone expert Clarence Ryan. Farmer and Ryan worked with local
sagebrush, which produce copious amounts of methyl jasmonate, an airborne
organic chemical that Ryan thought plants were using to ward off insect
herbivores. In their experiment, when damaged sagebrush leaves were put into
airtight jars with potted tomato plants, the tomatoes began producing
proteinase inhibitors — compounds that harm insects by disrupting their
digestion. Interplant communication is real, they said in a
1990 paper[18]
“If such
signaling is widespread in nature it could have profound ecological
significance.”
During the next
decade, evidence grew. It turns out almost every green plant that’s been
studied releases its own cocktail of volatile chemicals, and many species
register and respond to these plumes. For example, the smell of cut grass —
a blend of alcohols, aldehydes, ketones and esters — may be pleasant to us
but to plants signals danger on the way. Heil has found that
when wild-growing lima beans are exposed to volatiles from other lima bean
plants being eaten by beetles, they grow faster and resist attack. Compounds
released from damaged plants prime the defenses of corn seedlings,
so that they later mount a more effective counterattack against beet
armyworms. These signals seem to be a universal language: sagebrush induces
responses in tobacco; chili peppers and lima beans respond to cucumber
emissions, too.
Plants can
communicate with insects as well, sending airborne messages that act as
distress signals to predatory insects that kill herbivores. Maize attacked
by beet armyworms releases a cloud of volatile chemicals that
attracts wasps to lay eggs in the caterpillars’ bodies. The emerging picture
is that plant-eating bugs, and the insects that feed on them, live in a
world we can barely imagine, perfumed by clouds of chemicals rich in
information. Ants, microbes, moths, even hummingbirds and tortoises (Farmer)
all detect and react to these blasts.
Rather
than using the vascular system to send messages across meters-long
distances, maybe plants release volatile chemicals as a faster, smarter way
to communicate with themselves. Other plants can then monitor these puffs of
airborne data. Bolstering this theory, most of these chemical signals seem
to travel no more than 50 to 100 centimeters, at which range a plant would
mostly be signaling itself.
Lipids
influence multiple stages of plant–pathogen interactions including
communication between the host and the microbe, activation and
implementation of plant defenses, and the pathogen life cycle[46].
Some pathogens recognize plant lipid‐derived signals to identify an
appropriate host. Other pathogens depend on the host for lipids as essential
molecules or as developmental signals. In contrast, plants have evolved
mechanisms to recognize microbial lipids and this can lead to elicitation of
defense responses. In several cases, lipid modifications target plant
signaling proteins and microbial elicitors to plant cell membranes where
defense signaling is initiated. The membrane also provides a reservoir from
which biologically active signaling lipids, or their precursors, are
released by a variety of hydrolytic enzymes. A large number of
lipid‐modifying enzymes are involved in the synthesis of signaling lipids.
This chapter focuses on progress made in recent years on lipids, lipid
signaling, lipid‐modifying enzymes and
lipid‐transfer proteins that influence the outcome of plant–pathogen
interactions.[46]
Parts
of the signal transduction pathway for lipid remodeling have been studied[12,24,22,48].
However, detailed metabolite analysis regarding lipid remodeling has been
limited. Previous studies have thus far focused only on several well-known
lipid classes[58] because
of the difficulties associated with the comprehensive analysis of plant
lipids, which consist of a wide variety of hydrophobic chemicals. In this
study, we applied untargeted metabolomic analysis, which allows
comprehensive chemical metabolic phenotyping of cells,
Plant
lipid droplets are found in seeds and in post-embryonic tissues. Lipid
droplets in seeds have been intensively studied, but those in post-embryonic
tissues are less well characterized. Although known by a variety of names,
here we will refer to all of them as lipid bodies (LBs). LBs are unique
spherical organelles which bud off from the endoplasmic reticulum, and are
composed of a single phospholipid (PL) layer enclosing a core of
triacylglycerides. Although initially viewed as simple stores for energy and
carbon, the emerging view is that LBs also function in cytoplasmic
signaling, with the minor LB proteins caleosin and steroleosin in a
prominent role. They appear to function in dormancy release by
reconstituting cell-cell signaling paths.
Most
land plants are able to form partnerships with certain fungi – known as
arbuscular mycorrhiza fungi – that live in the soil[27].To
exchange nutrients, the fungi grow into the roots of the plant and form
highly branched structures known as arbuscules inside plant cells. Due to
the difficulties of studying this partnership, it has long been believed
that plants only provide sugars to the fungus. However, it has recently been
discovered that these fungi lack important genes required to make molecules
known as fatty acids. Therefore, these results raise the possibility that
the plant may provide the fungus with some of the fatty acids the fungus
needs to grow and the plant-fungi communications are governed via small
lipid signaling molecules.
References
1. Al
Mazroa MA, Memish ZA, AlWadey AM. Pandemic influenza A(H1N1) in Saudi
Arabia: Description of the first one hundred cases. Ann
Saudi Med. 2010;30:11–4. [PMC
free article] [PubMed] [Google
Scholar]
2. Alraddadi
BM, Watson JT, Almarashi A, Abedi GR, Turkistani A, Sadran M, et al. Risk
factors for primary middle east respiratory syndrome coronavirus illness in
humans, Saudi Arabia, 2014. Emerg Infect
Dis. 2016;22:49–55. [PMC
free article] [PubMed] [Google
Scholar]
3. Alsahafi
AJ, Cheng AC. The epidemiology of Middle East respiratory syndrome
coronavirus in the Kingdom of Saudi Arabia, 2012-2015. Int
J Infect Dis. 2016;45:1–4. [PMC
free article] [PubMed] [Google
Scholar]
4. Appuhamy
RD, Beard FH, Phung HN, Selvey CE, Birrell FA, Culleton TH, et al. The
changing phases of pandemic (H1N1) 2009 in Queensland: An overview of public
health actions and epidemiology. Med J
Aust. 2010;192:94–7. [PubMed] [Google
Scholar]
5. Assiri
A, Al-Tawfiq JA, Al-Rabeeah AA, Al-Rabiah FA, Al-Hajjar S, Al-Barrak A, et
al. Epidemiological, demographic, and clinical characteristics of 47 cases
of Middle East respiratory syndrome coronavirus disease from Saudi Arabia: A
descriptive study. Lancet Infect Dis. 2013;13:752–61. [PMC
free article] [PubMed] [Google
Scholar]
6.
Azekawa
S, Namkoong H, Mitamura K, Kawaoka Y, Saito F.
Co-infection with SARS-CoV-2 and influenza A virus.
IDCases. 2020 Apr 21;20:e00775.
doi: 10.1016/j.idcr.2020.e00775. eCollection 2020.PMID: 32368495 Free
PMC article.
7. Balkhy
HH, Alenazi TH, Alshamrani MM, Baffoe-Bonnie H, Arabi Y, Hijazi R, et al.
Description of a hospital outbreak of middle east respiratory syndrome in a
large tertiary care hospital in Saudi Arabia. Infect
Control Hosp Epidemiol. 2016;37:1147–55. [PMC
free article] [PubMed] [Google
Scholar]
8. Balla M, Merugu GP, Patel M, Koduri NM, Gayam V, Adapa S,
et al. COVID-19, modern pandemic: a systematic review from a front-line
health care providers’ perspective.
J
Clin Med Res
2020;12(4):215–229.
9.
Bhatraju PK, Ghassemieh BJ, Nichols M, Kim R, Jerome KR, Nalla AK, et al.
Covid-19 in critically ill patients in the Seattle region
–
case series. N Engl J Med 2020,
doi:http://dx.doi.org/10.1056/NEJMoa2004500.
10. Bialek
SR, Allen D, Alvarado-Ramy F, Arthur R, Balajee A, Bell D, et al. First
confirmed cases of Middle East respiratory syndrome coronavirus (MERS-coV)
infection in the United States, updated information on the epidemiology of
MERS-coV infection, and guidance for the public, clinicians, and public
health authorities – May 2014. MMWR Morb
Mortal Wkly Rep. 2014;63:431–6. [PMC
free article] [PubMed] [Google
Scholar]
11.
Brundage JF, Shanks GD. Deaths from bacterial pneumonia
during 1918–19 influenza pandemic. Emerg Infect Dis 2008; 14:1193–1199.
CrossRef |
PubMed |
Web of Science® Times Cited: 118
12.
Byers J.A. Pheromone biosynthesis in the bark beetle, Ips paraconfusus,
during feeding or exposure to vapors of host plant precursors.
Insect Biochem. 1981; 11: 563-569
View in Article |
Scopus (65)
|
Crossref
|
Google Scholar
13. Centers
for Disease Control and Prevention. [Last accessed on 2017 Feb 21];CDC
Health Update: Swine Influenza A(H1N1) Update: Influenza Activity- United
States. Centers for Disease Control and Prevention; 29 September, 8
February, 2013. 2014 [Google
Scholar]
14. Centers
for Disease Control and Prevention. Influenza (Flu). Available from
https://www.cdc.gov/flu/about/burden/index.html
(accessed 11 April 2020).
15. Dee
S, Jayathissa S. Clinical and epidemiological characteristics of the
hospitalised patients due to pandemic H1N1 2009 viral infection: Experience
at Hutt hospital, New Zealand. N
Z Med J. 2010;123:45–53. [PubMed] [Google
Scholar]
16. Delaney
JW, Fowler RA. 2009 influenza A(H1N1): A clinical review. Hosp
Pract (1995) 2010;38:74–81. [PubMed] [Google
Scholar]
17. Domínguez-Cherit
G, Lapinsky SE, Macias AE, Pinto R, Espinosa-Perez L, de la Torre A, et al.
Critically ill patients with 2009 influenza A(H1N1) in Mexico. JAMA. 2009;302:1880–7. [PubMed] [Google
Scholar]
18.
Farmer, E.E. and Ryan,
C.A. Interplant
communication: airborne methyl jasmonate induces synthesis of proteinase
inhibitors in plant leaves.
PNAS,
1990; 87 (19), 7713-7716; https://doi.org/10.1073/pnas.87.19.7713
19. File
TM. Community-acquired pneumonia. Lancet. 2003;362:1991–2001. [PMC
free article] [PubMed] [Google
Scholar]
20. Galván
JM, Rajas O, Aspa J. Review of non-bacterial infections in respiratory
medicine: Viral pneumonia. Arch
Bronconeumol. 2015;51:590–7. [PMC
free article] [PubMed] [Google
Scholar]
21. García-García
ML, Calvo C, Pozo F, Villadangos PA, Pérez-Breña P, Casas I, et al. Spectrum
of respiratory viruses in children with community-acquired pneumonia. Pediatr
Infect Dis J. 2012;31:808–13. [PubMed] [Google
Scholar]
20. Glezen
WP. Serious morbidity and mortality associated with influenza epidemics. Epidemiol
Rev. 1982;4:25–44. [PubMed] [Google
Scholar]
21.
Hers JF, Masurel N, Mulder J. Bacteriology and histopathology of the respiratory tract and lungs
in fatal Asian influenza. Lancet 1958; 2:1141–1143.
CrossRef | PubMed | CAS | Web
of Science® Times Cited: 126
22.
Goldstein J.L., Brown M.S. Regulation of the mevalonate pathway.
Nature. 1990; 343: 425-430
View in Article |
Scopus (4156)
|
PubMed
|
Crossref
|
Google Scholar
23.
Human infection with new
influenza A (H1N1) virus: clinical observations from Mexico and other
affected countries, May 2009. Wkly Epidemiol Rec 2009; 84:185–189.
PubMed
24.
Hunt D.W.A. et al. Sex-specific production of ipsdienol and myrcenol by Dendroctonus
ponderosae (Coleoptera: Scolytidae) exposed to myrcene vapors.
J. Chem. Ecol. 1986; 12: 1579-1586
View in Article |
Scopus (33)
|
PubMed
|
Crossref
|
Google Scholar
25.
Jain S, Kamimoto L, Bramley AM et al. Hospitalized patients with 2009 H1N1 influenza in the United
States, April–June 2009. N Engl J Med 2009; 361:1935–1944.
CrossRef |
PubMed |
CAS | Web
of Science® Times Cited: 751
26. John Hopkins University of Medicine Coronavirus Resource
Center. Available from
https://coronavirus.jhu.edu/map.html
(accessed 11 April 2020).
27.
Keymer
PimprikarWewer
V.Huber
C.Brands
M.
S.L.
Delaux
P.-M.Klingl
V.von
Röpenack-Lahaye
E.
T.L.
Eisenreich
W.
Dörmann
P.
Parniske
M.
GutjahrC.
eLife 2017;6:e29107 DOI: 10.7554/eLife.29107
https://elifesciences.org/articles/29107
28.
Khodamoradi
Z, Moghadami M, Lotfi M.Co-infection
of Coronavirus Disease 2019 and Influenza A: A Report from Iran.
Arch Iran Med. 2020 Apr 1; 23(4):239-243.
doi: 10.34172/aim.2020.04.PMID: 32271596
29.
Klein
et al.
(2016) The frequency of influenza and
bacterial coinfection: a systematic review and meta-analysis. Influenza and
Other Respiratory Viruses 10(5), 394–403
30.
Konala VM, Adapa S, Gayam V, Naramala
S, Duggubati SR, Kammari CB, Chenna A. Co-infection with influenza A and
COVID-19. EJCRIM
2020;
7: doi:10.12890/2020_001656.
31. Lan
YC, Su MC, Chen CH, Huang SH, Chen WL, Tien N, et al. Epidemiology of
pandemic influenza A/H1N1 virus during 2009-2010 in Taiwan. Virus Res. 2013;
177:46–54. [PubMed] [Google
Scholar]
32.
Lian J, Jin X, Hao S, Cai H, Zhang S, Zheng L, et al. Analysis of
epidemiological and clinical features in older patients with corona virus
disease 2019 (COVID-19) out of Wuhan. Clin Infect Dis 2020, doi:http://dx.doi.org/10.1093/cid/ciaa242
pii: ciaa242.
33.
Lee L.N., Dias P., Han D., et al.: A mouse model of lethal synergism between
influenza virus and Haemophilus influenzae. Am J Pathol 176: 800-811
34.
Lindsay MI Jr, Herrmann EC Jr, Morrow GW Jr, Brown AL Jr. Hong Kong influenza: clinical,
microbiologic, and pathologic features in 127 cases. JAMA 1970; 214:1825–1832.
CrossRef | PubMed | Web
of Science® Times Cited: 74
35. Louie
JK, Acosta M, Winter K, Jean C, Gavali S, Schechter R, et al. Factors
associated with death or hospitalization due to pandemic 2009 influenza
A(H1N1) infection in California. JAMA. 2009;302:1896–902. [PubMed] [Google
Scholar]
36.
McCullers,
J. A. Do specific virus–bacteria
pairings drive clinical outcomes of pneumonia?
Microbiology and Infection,
volume
19, Issue 2 (2013), pp
113–118
37.
Minhas V., Harvey R.M., McAllister L.J., Seemann T., Syme A. E., Baines S. L.,
Paton J. C., Trappetti C.
Capacity To
Utilize Raffinose Dictates Pneumococcal Disease Phenotype.
MBIO,
e02596-18
|
DOI: 10.1128/mBio.02596-18
|
https://mbio.asm.org/content/10/1/e02596-18
38.
Morens DM, Taubenberger JK, Fauci AS. Predominant role of bacterial pneumonia as a cause of death in
pandemic influenza: implications for pandemic influenza preparedness. J Infect Dis 2008; 198:
962–970.
CrossRef |
PubMed | Web
of Science® Times Cited: 306
39. Novel
Swine-Origin Influenza A(H1N1) Virus Investigation Team. Dawood FS, Jain S,
Finelli L, Shaw MW, Lindstrom S, et al. Emergence of a novel swine-origin
influenza A(H1N1) virus in humans. N Engl J
Med. 2009;360:2605–15. [PubMed] [Google
Scholar]
40.
Petersdorf RG, Fusco JJ, Harter DH, Albrink WS. Pulmonary infections
complicating Asian influenza. AMA Arch Intern Med 1959; 103:262–272.
CrossRef | PubMed | CAS | Web
of Science® Times Cited: 64
41.
Rodriguez JA, Rubio-Gomez H, Roa
AA, Miller N, Eckardt PA.
Co-Infection with SARS-COV-2 and Parainfluenza in a young adult patient with
pneumonia: Case Report.
IDCases. 2020 Apr 20;20:e00762. doi:
10.1016/j.idcr.2020.e00762. eCollection
2020.PMID: 32368493 Free
PMC article.
42. Ruuskanen
O, Lahti E, Jennings LC, Murdoch DR. Viral pneumonia. Lancet. 2011;377:1264–75. [PMC
free article] [PubMed] [Google
Scholar]
43.
Sangil A, Calbo E, Robles A, Benet S, Viladot
ME, Pascual V, et al. Aetiology of community-acquired pneumonia among adults
in an H1N1 pandemic year: The role of respiratory viruses. Eur
J Clin Microbiol Infect Dis. 2012;31:2765–72. [PMC
free article] [PubMed] [Google
Scholar]
44.
Schwarzmann SW, Adler JL, Sullivan RJ Jr, Marine WM. Bacterial pneumonia during the
Hong Kong influenza epidemic of 1968–1969. Arch Intern Med 1971; 127:1037–1041.
CrossRef | PubMed | CAS | Web
of Science® Times Cited: 202
45. Serfling
RE, Sherman IL, Houseworth WJ. Excess pneumonia-influenza mortality by age
and sex in three major influenza A2 epidemics, United States, 1957-58, 1960
and 1963. Am J Epidemiol. 1967;86:433–41. [PubMed] [Google
Scholar]
46.
Shah J.,
Chaturvedi R. 10 Lipid Signals in Plant–Pathogen Interactions. Annual Plant
Reviews book series, (2018) Volume 34: Molecular Aspects of Plant Disease Resistance.
https://doi.org/10.1002/9781119312994.apr0370
47.
Shuhei Azekawa, Ho Namkoong, Keiko Mitamura, Yoshihiro Kawaoka, Fumitake
Saito Co-infection with SARS-CoV-2 and influenza
A virus.
IDCases,
Volume 20, 2020, e00775;
https://doi.org/10.1016/j.idcr.2020.e00775
48.
Tittiger C. et al. Structure and
juvenile hormone-mediated regulation of the HMG-CoA reductase gene from the
Jeffrey pine beetle, Dendroctonus jeffreyi.
Mol. Cell. Endocrinol. 2003; 199: 11-21
View in Article |
Scopus (42)
|
PubMed
|
Crossref
|
Google Scholar
49.
Touzard-Romo F, Tapé C, Lonks
JR.
Co-infection with SARS-CoV-2 and Human Metapneumovirus.
R I Med J (2013). 2020 Mar
19;103(2):75-76.PMID: 32192233
50. World
Health Organization. “Acute Respiratory
Update”. [Last accessed on 2017 Feb
21]. Available from: http://www.who.int/vac-Cine_Research/diseases/ari/en/index5 .
51. World
Health Organization. Distribution of Con- Firmed Cases of Influenza A(H1N1)
by Age. WHO
European Region. [Last accessed on 2009 Apr/May]. Available from: http://www.euro.who.int/influenza/AH1N0090508_5 .
52. World
Health Organization. Middle East
respiratory Syndrome Coronavirus (MERS-CoV) [Last
accessed on 2017 Feb 21]. Available from: http://www.who.int/emergencies/mers-cov/en .
53.
Coinfection of Influenza Virus and Severe Acute Respiratory Syndrome
Coronavirus 2 (SARS-COV-2).
Wu D, Lu J, Ma X, Liu Q, Wang D, Gu Y, Li Y, He W.
Pediatr Infect Dis J. 2020
Jun;39(6):e79. doi:
10.1097/INF.0000000000002688.PMID: 32287051
Free PMC article.
54.
Wu X, Cai
Y, Huang X, Yu X, Zhao L, Wang F, et al. Co-infection with SARS-CoV-2 and
Influenza A Virus in Patient with Pneumonia, China. Emerg Infect Dis.
2020;26(6):1324-1326. https://dx.doi.org/10.3201/eid2606.200299
55. Xing Q, Li GJ, Xing
YH, Chen T, Li WJ, Ni W, et al. Precautions are needed for COVID-19 patients
with coinfection of common respiratory pathogens. Available from
http://dx.doi. org/10.2139/ssrn.3550013 (accessed 17 April
2020).
56.
Zhou F, Yu T, Du R, Fan G, Liu Y, Liu Z, et al. Clinical course and risk
factors for mortality of adult inpatients with COVID-19 in Wuhan, China: a
retrospective cohort study. Lancet 2020;395(10229):1054–62, doi:http://dx.doi.org/10.1016/
S0140-6736(20)30566-3.
57.
https://bio.libretexts.org/Bookshelves/Biochemistry/Book%3A_Biochemistry_Online_
(Jakubowski)/01%3A_LIPID_STRUCTURE/1.6%3A_Lipids_and_Signaling
58.
Dickens J.C. Green leaf volatiles enhance aggregation pheromone of the boll
weevil Anthonomus grandis. Entomol. Exp.
appl. 1989; 52: 191-203
View in Article | Scopus
(99)
| Crossref | Google
Scholar
59.
Malyarenko TV, Kicha AA, Malyarenko OS et
al. New conjugates of polyhydroxysteroids with long-chain
fatty acids from the deep-water far eastern starfish Ceramaster
patagonicus and their anticancer activity. Mar.
Drugs 18(5), 260 (2020).
60.
H. Clifford Lane, Anthony S. Fauci. (2020) Research in the Context of a
Pandemic. N
Engl J Med. July
17, 2020; DOI: 10.1056/NEJMoa2021436
61.
Martinez-Micaelo N, González-Abuín N, Ardèvol A, Pinent M, Blay MT (2012).
"Procyanidins and inflammation: Molecular targets and health implications". BioFactors. 38 (4):
257–265. doi:10.1002/biof.1019. PMID 22505223
62.
Ardalani, Hamidreza; Hadipanah, Amin; Sahebkar,
Amirhossein (27 December 2019). "Medicinal plants in the treatment of peptic
ulcer disease: A review". Mini-Reviews in Medicinal Chemistry. 20 (8):
662–702. doi:10.2174/1389557520666191227151939. PMID 31880244.
63. Izzi V,
Masuelli L, Tresoldi I, Sacchetti P, Modesti A, Galvano F, Bei R (2012).
"The effects of dietary flavonoids on the regulation of redox inflammatory
networks". Frontiers in Bioscience. 17 (7): 2396–2418. doi:10.2741/4061. PMID 22652788
64.
Gomes A, Couto D, Alves A, Dias I, Freitas M,
Porto G, Duarte JA, Fernandes E (2012). "Trihydroxyflavones with antioxidant
and anti-inflammatory efficacy". BioFactors. 38 (5): 378–386. doi:10.1002/biof.1033. PMID 22806885
Additional
Literature
Landolt P.J, Phillips T.W. Host plant
influences on sex pheromone behavior of phytophagous insects.
Annu. Rev. Entomol. 1997; 42: 371-391
View in Article |
Scopus (252)
|
PubMed
|
Crossref
|
Google Scholar
Nishida R. Sequestration of defensive
substances from plants by Lepidoptera. Annu.
Rev. Entomol. 2002; 47: 57-92
View in Article |
Scopus (377)
|
PubMed
|
Crossref
|
Google Scholar
Conner W.F et al. Courtship pheromone
production and body size as correlates of larval diet in males of the
arctiid moth, Utethisa ornatrix. J.
Chem. Ecol. 1990; 16: 543-552
View in Article |
Scopus (73)
|
PubMed
|
Crossref
|
Google Scholar
Eisner T, Meinwald J. The chemistry of
sexual selection. Proc. Natl. Acad. Sci. U.
S. A. 1995; 92: 50-55
View in Article |
Scopus (156)
|
PubMed
|
Crossref
|
Google Scholar
Hartmann T. Chemical ecology of
pyrrolizidine alkaloids. Planta. 1999; 207: 483-495
View in Article |
Scopus (201)
|
Crossref
|
Google Scholar
Lindigkeit R. et al. The two faces of
pyrrolizidine alkaloids: the role of the tertiary amine and its N-oxide in
chemical defense of insects with acquired plant alkaloids.
Eur. J. Biochem. 1997; 245: 626-636
View in Article |
Scopus (102)
|
PubMed
|
Crossref
|
Google Scholar
Winter C.K., Segall H.J. Metabolism of
pyrrolizidine alkaloids. in: Cheeke P.R Toxicants
of Plant Origin. Vol. 1. CRS Press, Boca
Raton, FL, USA1989: 23-40
View in Article |
Google Scholar
Naumann C. et al. Evolutionary recruitment
of a flavin-dependent monooxygenase for the toxification of host-plant
acquired pyrrolizidine alkaloids in the alkaloid-defended arctiid moth Tyria
jacobaeae. Proc. Natl. Acad. Sci. U. S.
A. 2002; 99: 6085-6090/
View in Article |
Scopus (87)
|
PubMed
|
Crossref
|
Google Scholar
Krasnoff S.B., Dussourd D.E.
Dihydropyrrolizine attractants for arctiid moths that visit plants
containing pyrrolizidine alkaloids. J. Chem.
Ecol. 1989; 15: 47-60
View in Article |
Scopus (44)
|
PubMed
|
Crossref
|
Google Scholar
Schulz S. Insect–plant interactions.
Metabolism of plant compounds to pheromones and allomones by Lepidoptera and
leaf beetles.
Eur. J. Org. Chem. 1998; : 13-20
View in Article |
Scopus (56)
|
Crossref
|
Google Scholar
Hartmann T et al. Are insect-synthesized
retronecine esters (creatonotines) the precursors of the male courtship
pheromone in the arctiid moth Estigmene acrea?.
J. Chem. Ecol. 2003; 29: 2603-2608
View in Article |
Scopus (22)
|
PubMed
|
Crossref
|
Google Scholar
Dressler R.L. Biology of the orchid bees
(Euglossini). Annu. Rev. Ecol. Syst. 1982; 13: 373-394
View in Article
|
Crossref
|
Google Scholar
Raina A.K. et al. Chemical signals from
host plant and sexual behavior in a moth.
Science. 1992; 255: 592-594
View in Article |
Scopus (73)
|
PubMed
|
Crossref
|
Google Scholar
Byers J.A. Pheromone biosynthesis in the
bark beetle, Ips paraconfusus, during feeding or exposure to vapors
of host plant precursors. Insect Biochem. 1981; 11: 563-569
View in Article |
Scopus (65)
|
Crossref
|
Google Scholar
Hunt D.W.A et al. Sex-specific production
of ipsdienol and myrcenol by Dendroctonus ponderosae (Coleoptera:
Scolytidae) exposed to myrcene vapors. J.
Chem. Ecol. 1986; 12: 1579-1586
View in Article |
Scopus (33)
|
PubMed
|
Crossref
|
Google Scholar
Renwick J.A.A et al. Selective production
of cis and trans-verbenol from (−)- and (+)-α-pinene by a
bark beetle. Science. 1976; 191: 199-201
View in Article |
Scopus (123)
|
PubMed
|
Crossref
|
Google Scholar
Feyereisen R. Insect P450 enzymes.
Annu. Rev. Entomol. 1999; 44: 507-533
View in Article |
Scopus (646)
|
PubMed
|
Crossref
|
Google Scholar
Seybold S.J et al. De novo biosynthesis
of the aggregation pheromone components ipsenol and ipsdienol by the pine
bark beetles Ips paraconfusus Lanier and Ips pini (Say)
(Coleoptera: Scolytidae). Proc. Natl. Acad.
Sci. U. S. A. 1995; 92: 8393-8397
View in Article |
Scopus (100)
|
PubMed
|
Crossref
|
Google Scholar
Tillman J.A et al. Endocrine regulation of
the novo aggregation pheromone biosynthesis in the pine engraver Ips
pini (Say) (Coleoptera: Scolytidae).
Insect Biochem.
Mol. Biol. 1998; 28: 705-715
View in Article |
Scopus (65)
|
Crossref
|
Google Scholar
Barkawi L.S et al. Frontalin: de novo biosynthesis
of an aggregation pheromone component by Dendroctonus spp. bark
beetles (Coleoptera: Scolytidae). Insect
Biochem. Mol. Biol. 2003; 33: 773-788
View in Article |
Scopus (47)
|
PubMed
|
Crossref
|
Google Scholar
Ivarsson P, Birgersson G. Regulation and
biosynthesis of pheromone components in the double spined bark beetle Ips
duplicatus (Coleoptera: Scolytidae). J.
Insect Physiol. 1995; 41: 833-849
View in Article |
Scopus (28)
|
Crossref
|
Google Scholar
Seybold S.J., Tittiger C. Biochemistry and
molecular biology of de novo isoprenoid pheromone production in the
Scolytidae. Annu. Rev. Entomol. 2003; 48: 425-453
View in Article |
Scopus (85)
|
PubMed
|
Crossref
|
Google Scholar
Tittiger C et al. Structure and juvenile
hormone-mediated regulation of the HMG-CoA reductase gene from the Jeffrey
pine beetle, Dendroctonus jeffreyi.
Mol. Cell. Endocrinol. 2003; 199: 11-21
View in Article |
Scopus (42)
|
PubMed
|
Crossref
|
Google Scholar
Goldstein J.L., Brown M.S. Regulation of
the mevalonate pathway. Nature. 1990; 343: 425-430
View in Article |
Scopus (4156)
|
PubMed
|
Crossref
|
Google Scholar
Dickens J.C. Green leaf volatiles enhance
aggregation pheromone of the boll weevil Anthonomus grandis.
Entomol. Exp. Appl. 1989; 52: 191-203
View in Article |
Scopus (99)
|
Crossref
|
Google Scholar
Jaffe K. et al. Chemical ecology of the
palm weevil Rhynchophorus palmarum (L.) (Coleoptera:
Curculionidae): attraction to host plants and to a male-produced aggregation
pheromone. J. Chem. Ecol. 1993; 19: 1703-1720
View in Article |
Scopus (86)
|
PubMed
|
Crossref
|
Google Scholar
Rochat D. et al. Identification of
pheromone synergists in American weevil, Rhynchophorus palmarus,
and attraction of related Dynamis borassi.
J. Chem. Ecol. 2000; 26: 155-187
View in Article |
Scopus (67)
|
Crossref
|
Google Scholar
McNeil J.N., Delisle J. Host plant pollen
influences calling behavior and ovarian development of the sunflower moth, Homeosoma
electellum. Oecologia. 1989; 80: 201-205
View in Article |
Google Scholar
|
Hendrikse A., Vos-Bunnemyer E. Role of host
plant stimuli in sexual behavior of small ermine moths (Yponomeuta).
Ecol. Entomol. 1987; 12: 363-371
View in Article |
Scopus (34)
|
Crossref
|
Google Scholar
Landolt P.J., Heath R.R. Sexual role
reversal in mate-finding strategies of the cabbage looper moth.
Science. 1990; 249: 1026-1028
View in Article |
Scopus (46)
|
PubMed
|
Crossref
|
Google Scholar
Nakamuta K. et al. Increase of trap catches
by a combination of male sex pheromones and floral attractant in longhorn
beetle, Anaglyptus subfasciatus. J.
Chem. Ecol. 1997; 23: 1635-1640
View in Article |
Scopus (41)
|
Crossref
|
Google Scholar
Erbilgin N., Raffa K.F. Effects of host
tree species on attractiveness of tunnelling pine engravers, Ips pini (Coleoptera:
Scolytidae), to conspecifics and insect predators.
J. Chem. Ecol. 2000; 26: 823-840
View in Article |
Scopus (38)
|
Crossref
|
Google Scholar
Renwick J.A.A., Vité J.P. Bark beetle
attractants: mechanism of colonization by Dendroctonus frontalis.
Nature. 1969; 224: 1222-1223
View in Article |
Scopus (99)
|
Crossref
|
Google Scholar
Reinecke A. et al. The scent of food and
defense: green leaf volatiles and toluquinone as sex attractant mediate mate
finding in the European cockchafer Melolontha melolontha.
Ecol. Lett. 2002; 5: 257-263
View in Article |
Scopus (41)
|
Crossref
|
Google Scholar
Reddy G.V.P et al. Olfactory responses of Plutella
xylostella natural enemies to host pheromone, larval frass, and green
leaf volatiles. J. Chem. Ecol. 2002; 28: 131-143
View in Article |
Scopus (127)
|
PubMed
|
Crossref
|
Google Scholar
Reddy G.V.P., Guerrero A. Behavioral
responses of the diamondback moth, Plutella xylostella, to green
leaf volatiles of Brassica oleracea subsp. capitata.
J. Agric. Food Chem. 2000; 48: 6025-6029
View in Article |
PubMed
|
Crossref
|
Google Scholar
Hayes J.L. et al. Repellent properties of
the host compound 4-allylanisole to the southern pine beetle.
J. Chem. Ecol. 1994; 20: 1595-1615
View in Article |
Scopus (55)
|
PubMed
|
Crossref
|
Google Scholar
Dickens J.C. et al. Green leaf volatiles
interrupt aggregation pheromone response in bark infesting pines.
Experientia. 1992; 48: 523-524
View in Article |
Scopus (91)
|
Crossref
|
Google Scholar
De Groot P., MacDonald L.M.
Green leaf volatiles inhibit response of red pine cone beetle Conophthorus
resinosae (Coleoptera: Scolytidae) to a sex pheromone.
Naturwissenschaften. 1999; 86: 81-85
View in Article |
Scopus (23)
|
Crossref
|
Google Scholar
Poland T.M., Haack R.A. Pine shoot beetle, Tomicus
piniperda (Col., Scolytidae), responses to common green leaf volatiles.
J. Appl. Entomol.
2000; 124: 63-69
View in Article |
Scopus (40)
|
Crossref
|
Google Scholar
Huber D.P.W., Borden J.H. Angiosperm bark
volatiles disrupt response of Douglas-fir beetle, Dendroctonus
pseudotsugae, to attractant-baited traps.
J. Chem. Ecol. 2001; 27: 217-233
View in Article |
Scopus (53)
|
PubMed
|
Crossref
|
Google Scholar
Poland T.M. et al. Green leaf volatiles
disrupt responses by the spruce beetle, Dendroctonus rufipennis,
and the western pine beetle, Dendroctonus brevicomis (Coleoptera:
Scolytidae) to attractant-baited traps. J.
Entomol. Soc. B.C. 1998; 95: 17-24
View in Article |
Google Scholar
|
Guerrero A. et al. Semiochemically induced
inhibition of behaviour of Tomicus destruens (Woll.) (Coleoptera:
Scolytidae). Naturwissenschaften. 1997;
84:
155-157
View in Article |
Scopus (38)
|
Crossref
|
Google Scholar
Thaler J.S. Jasmonate-inducible plant
defenses cause increased parasitism of herbivores.
Nature. 1999; 339: 686-688
View in Article |
Scopus (414)
|
Crossref
|
Google Scholar
De Moraes C.M. et al. Caterpillar-induced
nocturnal plant volatiles repel non-specific females.
Nature. 2001; 410: 577-580
View in Article |
Scopus (638)
|
PubMed
|
Crossref
|
Google Scholar
Erbilgin N., Raffa K.F. Modulation of
predator attraction to pheromones of two prey species by stereochemistry of
plant volatiles. Oecologia. 2001;
127: 444-453.
View in Article |
Scopus (66)
|
Crossref
|
Google Scholar
Raffa K.F., Klepzig K.D. Chiral escape of
bark beetles from predators responding to a bark beetle pheromone.
Oecologia. 1989; 80: 566-569
View in Article |
Scopus (68)
|
Crossref
|
Google Scholar
Agrawal A.A. Mechanisms, ecological
consequences and agricultural implications of tri-trophic interactions.
Curr. Opin. Plant Biol. 2000; 3: 329-335
View in Article |
Scopus (87)
|
PubMed
|
Crossref
|
Google Scholar
Takabayashi J., Dicke M. Plant–carnivore
mutualism through herbivore-induced carnivore attractants.
Trends Plant Sci. 1996; 1: 109-113
View in Article |
Scopus (362)
|
Abstract
|
Full Text PDF
|
Google Scholar
Tumlinson J.H. et al. How parasitic wasps
find their hosts. Sci. Am. 1993; 268: 100-106
View in Article |
PubMed
|
Crossref
|
Google Scholar
Paré P.W., Tumlinson J.H. Plant volatiles
as a defense against insect herbivores. Plant
Physiol. 1999; 121: 325-331
View in Article |
PubMed
|
Crossref
|
Google Scholar
Mattiacci L. et al. β-Glucosidase: an
elicitor of herbivore-induced plant odor that attracts host-searching
parasitic wasps. Proc. Natl. Acad. Sci. U. S.
A. 1995; 92: 2036-2040
View in Article |
Scopus (434)
|
PubMed
|
Crossref
|
Google Scholar
Alborn H.T. et al. An elicitor of plant
volatiles from beet armyworm oral secretion.
Science. 1997; 276: 945-949
View in Article |
Scopus (673)
|
Crossref
|
Google Scholar
Meiners T., Hilker M. Induction of plant
synomones by oviposition of a phytophagous insect.
J. Chem. Ecol. 2000; 26: 221-232
View in Article |
Scopus (151)
|
Crossref
|
Google Scholar
Doss R.P. et al. Bruchins: insect-derived
plant regulators that stimulate neoplasm formation.
Proc. Natl. Acad. Sci. U. S. A. 2000; 97: 6218-6223
View in Article |
Scopus (157)
|
PubMed
|
Crossref
|
Google Scholar
Baldwin I.T. et al. Merging molecular and
ecological approaches in plant-insect interactions.
Curr. Opin. Plant Biol. 2001; 4: 351-358
View in Article |
Scopus (148)
|
PubMed
|
Crossref
|
Google Scholar
Schittko U. et al. Eating the evidence? Manduca
sexta can not disrupt specific jasmonate induction in Nicotiana
attenuata by rapid consumption. Planta. 2000; 210: 343-346
View in Article |
Scopus (84)
|
PubMed
|
Crossref
|
Google Scholar
Baldwin I.T. An ecologically motivated
analysis of plant-herbivore interactions in native tobacco.
Plant Physiol. 2001; 127: 1449-1458
View in Article |
Scopus (177)
|
PubMed
|
Crossref
|
Google Scholar
Kahl H. et al. Herbivore-induced ethylene
suppresses a direct defense but not a putative indirect defense against an
adapted herbivore. Planta. 2000; 210: 336-342
View in Article |
Scopus (257)
|
PubMed
|
Crossref
|
Google Scholar
Voelckel C. et al. Herbivore-induced
ethylene burst reduces fitness costs of jasmonate- and oral
secretion-induced defenses in Nicotiana attenuata.
Oecologia. 2001; 127: 274-280
View in Article |
Scopus (39)
|
PubMed
|
Crossref
|
Google Scholar
Kessler A., Baldwin I.T. Defensive function
of herbivory-induced plant volatile emissions in nature.
Science. 2001; 291: 2141-2144
View in Article |
Scopus (1320)
|
PubMed
|
Crossref
| |
Google Scholar
Klein M.G. et al. Japanese beetle
(Coleoptera: Scarabeidae): response to synthetic sex attractants plus
phenethyl:eugenol. J. Chem. Ecol. 1981; 7: 1-7
View in Article |
Scopus (25)
|
PubMed
|
Crossref
|
Google Scholar
Lin H.L.P. et al. Synergism between
synthetic food odors and the aggregation pheromone for attracting Carpophilus
lugubris in the field (Coleoptera: Nitidulidae).
Environ. Entomol. 1992; 21: 156-159
View in Article |
Google Scholar
Gries G. et al. Ethyl propionate:
synergistic kairomone for African palm weevil, Rhynchophorus phoenicis L.
(Coleoptera: Curculionidae). J. Chem. Ecol. 1994; 20: 889-897
View in Article |
Scopus (45)
|
PubMed
|
Crossref
|
Google Scholar
Borden J.H. et al. Ethanol and α-pinene as
synergists for the aggregation pheromones of two Gnathotrichus species.
Can. J. Forest Res. 1980; 10: 290-292
View in Article |
Crossref
|
Google Scholar
Ochieng S.A. et al. Host plant volatiles
synergize responses of sex pheromone-specific olfactory receptor neurons in
male Helicoverpa zea. J. Comp.
Physiol. A. 2002; 188: 325-333
View in Article
|
Scopus (124)
|
Crossref
|
Google Scholar
Giblin-Davis R.M. et al. Optimization of
semiochemical-based trapping of Metamasius hemipterus sericeus (Olivier)
(Coleoptera: Curculionidae). J. Chem. Ecol. 1996; 22: 1389-1410
View in Article |
Scopus (41)
|
PubMed
|
Crossref
|
Google Scholar
Dowd P.F., Bartelt R.J. Host-derived
volatiles as attractants and pheromone synergists for driedfruit beetle, Carpophilus
hemipterus. J. Chem. Ecol. 1991; 17: 285-308
View in Article |
Scopus (33)
|
PubMed
|
Crossref
|
Google Scholar
Phillips T.W. et al. Behavioral responses
to food volatiles by two species of stored-product Coleoptera, Sitophilus
oryzae (Curculionidae) and Tribolium castaneum (Tenebrionidae).
J. Chem. Ecol. 1993; 19: 723-734
View in Article |
Scopus (92)
|
PubMed
|
Crossref
|
Google Scholar
Rochat D. et al. Chemical ecology of palm
weevils, Rhynchophorus spp. (Coleoptera).
Oleagineux Paris. 1993; 48: 225-236
View in Article |
Google Scholar
Giblin-Davis R.M. et al. Field response of Rhychophorus
cruentatus (Coleoptera: Curculionidae) to its aggregation pheromone and
fermenting plant volatiles. Florida
Entomologist. 1994; 77: 164-177
View in Article |
Scopus (53)
|
Crossref
|
Google Scholar
Bartelt R.J. et al. Male produced
aggregation pheromone of Carpophilus freemani (Coleoptera:
Nitidulidae). J. Chem. Ecol. 1993; 19: 107-118
View in Article |
Scopus (35)
|
PubMed
|
Crossref
|
Google Scholar
Petroski R.J. et al. Male-produced
aggregation pheromone of Carpophilus obsoletus (Coleoptera:
Nitidulidae). J. Chem. Ecol. 1994; 20: 1483-1493
View in Article |
Scopus (18)
|
PubMed
|
Crossref
|
Google Scholar
Hallett R.H. et al. Pheromone trapping
protocols for the Asian palm weevil, Rhynchophorus ferrugineus (Coleoptera:
Curculionidae). Int. J. Pest Manage. 1999; 45: 231-237
View in Article |
Scopus (72)
|
Crossref
|
Google Scholar
|
Sudharto P.S. et al. Synergy between empty
oil palm fruit bunches and synthetic aggregation pheromone (ethyl
4-methyloctanoate) for mass trapping of Oryctes rhinoceros beetles
in the oil palm plantations in Indonesia. Cutting Edge Technologies for
Sustained Competitiveness: Proceedings of the 2001 PIPOC International Palm
Oil Congress. Malaysian Palm Oil Board, Kuala
Lumpur, Malaysia2000
View in Article |
Google Scholar
Muniappan, R. et
al. Field response of the two geographical isolates of New Guinea
sugarcane weevil, Rhabdoscelus obscurus (Boisduval) (Coleoptera:
Curculionidae) to their aggregation pheromone and host volatiles in Guam. Micronesica (in
press).
View in Article |
Google Scholar
Bartelt R.J. et al. Aggregation pheromones
in Drosophila borealis and Drosophila littoralis.
J. Chem. Ecol. 1988; 14: 1319-1327
View in Article |
Scopus (26)
|
PubMed
|
Crossref
|
Google Scholar
Light D.M. et al. Host-plant green
volatiles synergize the synthetic sex pheromones of the corn earworm and
codling moth (Lepidoptera). Chemoecology. 1993; 4: 145-152
View in Article |
Scopus (134)
|
Crossref
|
Google Scholar
Dickens J.C. et al. Detection and
deactivation of pheromone and plant odor components by the beet armyworm, Spodoptera
exigua (Hubner) (Lepidoptera: Noctuidae).
J. Insect Physiol. 1993; 39: 503-516
View in Article |
Scopus (61)
|
Crossref
|
Google Scholar
Hardie J. et al. The interaction of sex
pheromone and plant volatiles for field attraction of male bird-cherry
aphid, Rhopalosiphum padi.
Proceedings – Brighton Crop Protection Conference, Pests and Diseases. Vol.
3. BCPC Publications, Bracknell,
UK1994
View in Article |
Google Scholar
Phillips T.W. et al. Aggregation pheromone
of the deodar weevil, Pissodes nemorensis (Coleoptera:
Curculionidae): isolation and activity of grandisol and grandisal.
J. Chem. Ecol. 1984; 10: 1417-1423
View in Article |
Scopus (45)
|
PubMed
|
Crossref
|
Google Scholar
Landolt P.J. et al. Attraction of female
papaya fruit fly (Diptera: Tephritidae) to male pheromone and host fruit.
Environ. Entomol. 1992; 21: 1154-1159
View in Article |
Google Scholar
Dickens J.C. et al. Green leaf volatiles
enhance sex attractant pheromone of the tobacco budworm, Heliothis
virescens (Lepidoptera: Noctuidae).
Chemoecology. 1993; 4: 175-177
View in Article |
Scopus (72)
|
Crossref
|
Google Scholar
Conner W.F. et al. Precopulatory sexual
interaction in an arctiid moth (Utetheisa ornatrix): role of a
pheromone derived from dietary alkaloids.
Behav. Ecol. Sociobiol. 1981; 9: 227-235
View in Article |
Scopus (142)
|
Crossref
|
Google Scholar
Seybold S.J. et al. The biosynthesis of
coniferophagous bark beetle pheromones and conifer isoprenoids: evolutionary
perspective and synthesis. Can. Entomol. 2000; 132: 697-753
View
in Article |
Scopus (96)
|
Crossref
|
Google Scholar
Related
Articles
·
A Bidirectional Circuit Switch Reroutes
Pheromone Signals in Male and Female Brains.
Kohl et al.
CellDecember
19, 2013
In Brief
Full-Text
PDF
Open
Access
·
Murine Pheromone Proteins Constitute a
Context-Dependent Combinatorial Code Governing Multiple Social Behaviors.
Kaur et
al.
CellApril 24, 2014
In Brief
Full-Text
PDF
Open
Archive
·
Role of Polarized G Protein Signaling in
Tracking Pheromone Gradients.
McClure et al.
Developmental CellNovember
23, 2015
In Brief
|
Full-Text
PDF
Open Archive
·
Biochemical and genetic characterization of a
competence pheromone from B. subtilis.
Magnuson
et al.
CellApril
22, 1994.
In Brief
Full-Text
PDF
·
Yeast peptide pheromones, a-factor and
α-factor, activate a common response mechanism in their target cells.
Bender et
al.
CellDecember
26, 1986
In Brief
Full-Text
PDF
|